Abstract
Natural compounds from marine organisms have been rarely studied for their acetylcholinesterase inhibitory activities. The aim of this study was to isolate novel compounds with antiAChE activity from the venom of upside-down jellyfish Cassiopea andromeda Forskål, 1775. The compounds of the fractionated venom on gel filtration chromatography were identified by analyzing gas chromatography–mass spectroscopy data. The structure of the isolated compound that showed the most potent antiAChE activity in a docking study was elucidated by different spectral data, including 1H NMR and 13C NMR. Three compounds, including a neurosteroidal alkaloid androtoxin B, were identified from two venom fractions. This neurosteroidal alkaloid showed strong acetylcholinesterase inhibitory activity (IC50 2.24 ± 0.1 µM) compared with the reference standard, galantamine. The results obtained by a docking study demonstrated that Androtoxin B had close contact with two of the three amino acid residues of the catalytic triad of acetylcholinesterase gorge and was accommodated within a peripheral hydrophobic pocket composed of numerous aromatic site chains. In conclusion, the isolated neurosteroidal alkaloid from Cassiopea andromeda was a potent antiAChE agent with strong binding to both the catalytic and peripheral sites of acetylcholinesterase that correlated well with the experimental data. Further studies are required to determine whether androtoxin B could be a potential treatment for Alzheimer's disease.
Keywords Upside-down jellyfish; Antiacetylcholinesterase activity; Androtoxin B; Alzheimer's disease; Neurosteroidal alkaloid; GABA-A receptor
Introduction
Alzheimer's disease is a fatal progressive neurodegenerative disorder of the brain affecting 25 million people worldwide (Ferreira-Vieira et al., 2016). Today, it is called the silver tsunami of the 21st century (Sarkar et al., 2016). The accumulation of neurofibrillary tangles, the amyloid-beta plaques in the brain, the progressive loss of neurons in the hippocampus and cortex, and the progressive decline in memory, thinking, and language skills are characteristics of this complex disease (Ferreira-Vieira et al., 2016).
The degeneration of cholinergic neurons results in memory and attention deficits of Alzheimer's disease (Ferreira-Vieira et al., 2016). Three cholinesterase inhibitors, donepezil, galantamine, and rivastigmine, have shown some effects on mild to moderate Alzheimer's disease (Birks, 2006). Therefore, the search for effective bioactive compounds with acetylcholinesterase (AChE) inhibitory activities from natural sources to combat Alzheimer's disease is a hot topic.
Although a large number of AChE inhibitors from medicinal plants have been reported (Ahmed et al., 2013), natural compounds from marine organisms have been rarely studied for their AChE inhibitory activities (Beedessee et al., 2013). The AChE inhibitory activity of plastoquinones from Sargassum sagamianum (Choi et al., 2007); the acetone extracts of Padina gymnospora (Shanmuganathan et al., 2015); fucoxanthin, which is a marine carotenoid (Peng et al., 2011); avarol, which is a marine sesquiterpenoid hydroquinone isolated from Dysidea avara (Tommonaro et al., 2016); extracts from sponges collected in Mauritius waters (Beedessee et al., 2013); and halogenated sesquiterpenes derived from the Persian Gulf (Farrokhnia and Nabipour, 2014) have been studied.
Cassiopea andromeda (Forsskal 1775; Cnidaria: Scyphozoa: Rhizostomea) is a type of jellyfish that lives in shallow, tropical inshore marine waters and is usually associated with mangrove populations (Holland et al., 2004). This jellyfish is also called upside-down jellyfish because, unlike the stereotypical jellyfish, it occurs in an inverted form in intertidal sand or mudflats with their tentacles oriented toward the sky. Although the genus Cassiopea has a global distribution in the Western Atlantic and Indo-Pacific tropical areas, Cassiopea was not observed in the Persian Gulf until 2014. The alien C. andromeda has been suggested to arrive at the Persian Gulf in the pellagic stage by the ballast water of ships (Nabipour et al., 2015). Currently; an established population of this upside-down jellyfish is present in Nayband Lagoon, Assalouyeh, Iran.
Cassiopea andromeda captures its prey through nematocysts. Some of the toxic characteristics of C. andromeda have been investigated (Radwan et al., 2001; Ellithey et al., 2014; Nabipour et al., 2017). Nematocyst venom of this jellyfish exhibited dermonecrotic, vasopermeability-producing properties, and hemorrhaging effects (Radwan et al., 2001; Nabipour et al., 2017). The methanolic extract of C. andromeda produced potent inhibitory activity against HIV-1 protease (Ellithey et al., 2014). Recently, we showed that the C. andromeda venom fractions induced selective injury and cytochrome C release in mitochondria obtained from breast adenocarcinoma patients (Mirshamsi et al., 2017).
As part of our research on the isolation of bioactive molecules from C. andromeda, the AChE inhibitory activities of the extracted fractions and compounds of this upside-down jellyfish were evaluated using conventional and docking methods.
Materials and methods
Sampling and sample preparation
A number of thirty live specimens of Cassiopea andromeda Forskål, 1775 were collected by means of a trawl net, from the Nayband Bay in the northern part (27º 30′ S, 52º 35′ E) of Bushehr, Iran, in spring of 2014 (Fig. 1). The bloom mainly was located at in depths of 0.5–1.5 m, on a muddy-sand bottom in Lagoon, moreover their aggregations were including about 4–5 individuals in each m2 and different sizes, but typically more ranges between 6 and 15 cm in diameter. The identities of the species were verified by Professor B. Holland from the University of Hawaii (Nabipour et al., 2015).
Location map of the study area (a population of the Cassiopea andromeda jellyfish in Nayband Bay, Bushehr-Iran).
The project was permitted by the Medical Ethics Committee of Bushehr University of Medical Sciences and Health Services, Bushehr, Iran. All animal experiments were based on the National Ethical Guidelines for Animal Research in Iran (2005) under a project license, which was approved by the Animal Care and Use Committee of Bushehr University of Medical Sciences, Iran, according to Protocol: D/P/3758.
The separation of the tentacles was performed according to Bloom et al. (1998) method, with some modifications. Briefly, the tentacles were excised manually from specimens as soon as possible after capture by the trawl, directly placed into small glass containers filled with a one-third portion of seawater, and transported in ice bags to the Persian Gulf Marine Biotechnology Research Center laboratories in the Persian Gulf Biomedical Research Center, Bushehr University of Medical Sciences, Iran. At that time, the tentacles were homogenized by a Homogenizer (IKA, Germany), kept at 4 ºC for two days for the autolysis of the tissues and release of toxins (Burnett et al., 1992), and centrifuged (Eppendorf, Germany) at 12,000 × g for 15 min at 4 ºC to remove the sediments. The final supernatant was freeze-dried (Christ, UK) and kept at -80 ºC until use (Mustafa et al., 1995). The nematocysts of the tentacles (black arrows) are shown in Fig. 2.
Protein concentration and molecular weight determination
The protein concentration of the lyophilized crude venom (a sky bluish powder) was measured according to the Bradford method (Bradford, 1976) using an ultraviolet–visible (UV–Vis) spectrophotometer (Cecil Aquarius Double Beam Spectrophotometer, England). Bovine serum albumin (BSA) (Sigma, Germany), was used as the calibration standard protein at concentrations of (5, 10, 15, 20, 25 mg ml-1). The protein concentration expressed in unit of µg ml-1. Briefly, a total of 10 µl of freeze dried venom was subjected to sodium dodecyl sulfate-polyacrylamide gel electrophoresis (SDS-PAGE) at 25 ºC, according to the Laemmli method (Laemmli, 1970).
Resolving gel (pH 8): percentage of gel 12.5%; 3.1 ml acrylamide: bisacrylamide (30:0.8% w/v); 3 ml Tris–Cl pH: 8.8; 38 µl SDS 20%; 1.3 ml dH2O; 5 µl ammonium persulfate (APS) 10%; 7.5 ml tetramethylethylenediamine (TEMED).
Stacking gel (pH 6.8 ): percentage of stack 6%; 1 ml acryl: bisacryl (30: 0.8% w/v); 630 µl Tris–Cl pH 8.8, 1 M; 25 µl SDS 20%; 3.6 ml dH2O; 24 µl APS 10%; and 5 ml TEMED) (Brinkman and Burnell, 2007). Proteins were visualized using Coomassie® Brilliant blue R 250 (Sigma, Germany). The molecular weights were evaluated by comparison to BioRad markers (Precision Plus Protein® Standards, BioRad, USA) at the ranges of 225, 150, 102, 76.52, 38, 17, and 12 kDa. All other chemicals were purchased from Merck (Darmstadt, Germany).
AChE activity
The crude venom and its fractions were tested for their ability to inhibit AChE from electric eel (C2888 – Electrophorus electricus AChE, type V, 1000 units/mg protein, Sigma–Aldrich), according to the Ellman kinetic method modified by Worek et al. (1999). Hydrolysis rate was monitored by verifying the formation of the TNB- of DTNB at 436 nm during 3 min at intervals of 15 s, using the Cecil UV-VIS Spectrophotometer. For the blank, the buffer instead of enzyme solution was used. The enzyme activity was measured using the equation:
The percentage (%) inhibition was calculated as follows: (E - S)/E × 100, where “E” is the activity of the enzyme without test compounds and “S” is the activity of enzyme with test compounds. The experiment was done in triplicate and concentrations of the samples that inhibit the hydrolysis of the substrate by 50% (IC50) were determined by linear regression analysis between the inhibition percentages versus the extract concentration by the Excel program.
Gel filtration chromatography
Sephadex G-200 (Pharmacia, USA) gels were poured into a 60 cm × 2.5 cm diameter column. Elution was completed with a 0.05 M phosphate buffer (pH 7.2 at 4 ºC), and the flow rate was adjusted to 0.5 ml/min. About 200 mg of the crude venom of C. andromeda was dissolved in the phosphate buffer. After centrifugation at 12,000 × g for 15 min at 4 ºC, the supernatant was loaded on the Sephadex G-200. The toxins were eluted with the phosphate buffer, the 4 ml fractions were collected with a fraction collector apparatus, and protein absorbance was measured at 280 nm by a UV spectrophotometer (Cecil 7000 series, England) (Othman and Burnett, 1990).
Gas chromatography–mass spectroscopy (GC–MS) of the fractions
The lyophilized fractions (white color), were subjected to the 7890B Agilent Gas Chromatography–Mass Spectroscopy. Electron ionization (EI) mass spectra (scan range, m/z 50–500) were obtained using electrons with energy of 70 eV energy and filament emission of 0.5 mA. The GC separations were conducted using an HP-5MS UI column (30 m × 0.25 mm i.d., film thickness 0.5 µm). Helium was used as the carrier gas (flow: 0.8 ml min-1) for EI. The GC oven was temperature programmed at 5 ºC min-1 from 80 ºC after 3 min since the sample injection and held at 250 ºC for 10 min. The injection port of the gas chromatograph, transfer line, and ion source of 5977MSD were maintained at 240 ºC, 250 ºC, and 220 ºC, respectively. The separated compounds were identified by comparing them with the compound data from the National Institute of Standards and Technology (NIST MS database, 2014) library. The relative percent amount of each component was measured by comparing its average peak area to the total areas.
NMR analysis
The structure of the purified compound with the most potent anticholinesterase activity dissolved in DMSO was elucidated on the basis of the result of the 1H NMR (400 MHz) and 13C NMR (100 MHz) Bruker Avance 400.
Computational details
The binding affinities between the identified structures (in addition to galantamine as the control compound) and the active site of recombinant human AChE receptor were examined through docking studies using the AutoDock 4.2.6 suites ((Morris et al., 2009). Docking logs were analyzed in the graphical user interface of the AutoDock Tool (ADT) (Sanner, 1999). The crystal structure of rhAChE in the complex with (-)-galantamine was obtained from the Brookhaven protein data bank with PDB accession code ey6 (Cheung et al., 2012). Before starting the docking simulations, (-)-galantamine was separated from the complex. As the positions of the water molecules in the crystal structure of the enzymes were unlikely to be conserved, they were excluded before the docking studies (Pilger et al., 2001). H-atoms were added to the hAChE protein for correct tautomeric states of amino acid residues, and the non-polar hydrogens were combined. The Kollman United Atom charges and atomic solvation parameters were assigned to the protein and the Gasteiger charge to the ligands. All structures were converted to PDBQT format in ADT as required in the AutoDock calculations. The size of the grid plotted for specifying the search space was set to 70 × 70 × 70 Å3 and centered on the predicted cavities with a default grid point spacing of 0.375 Å. Pre-calculated grid maps, which store grids of interaction energy based on the interaction of the ligand atom with the receptor target, were obtained using AutoGrid. It computes the van der Waals energies at each single grid point. Additionally, the Lamarckian genetic algorithm (GA) was used with a population size of 150 dockings and 100 GA runs. Five million energy evaluations were used in the docking, and the crossover and mutation rates were run with default settings. The obtained lowest docked energy conformation of the ligand in the most populated cluster among all the 100 GA runs used for further studies. The outputs from AutoDock and images were generated with PyMOL (DeLano, 2002), and Accelerys ViewerLite 5.0a (2002).
Results and discussion
The protein concentration and SDS-PAGE analysis
The protein content of the crude venom, quantified by the Bradford method, was found to be 17.5 mg ml-1. The SDS-PAGE analysis with 12% acrylamide gel, revealed at least eleven prominent protein bands, with molecular masses ranging from 12 to 225 kDa. The main bands were located at the approximate molecular weights of 12, 17, 28, 34, 38, 43, 50, 53, 76, 102 and 225 kDa (Fig. 3). A number of minor bands were also observed around these regions.
SDS-PAGE (12% polyacrylamide gel stained with Coomassie brilliant blue) analysis of protein standard marker (M), Cassiopea andromeda venom sample (S).
AChE activity
Experimental data based on Ellman's spectroscopic method showed that the crude venom and fraction B exhibited proper AChE inhibitory activities. The IC50 of the crude venom, fractions A and B were 4.81 ± 0.25, 7.03 ± 0.012 and 2.24 ± 0.1 (µM) at 37 ºC, respectively.
Gel filtration chromatography
The crude venom was fractionated using gel filtration chromatography. As shown in Fig. 4, the chromatogram of C. andromeda crude venom on Sephadex G-200 revealed two fractions (fa and fb) with absorbance at 280 nm. The fa was called Androtoxin A (ATXA) and fb was called Androtoxin B (ATXB). The fractions were collected on 4 ml volumes, freeze dried, and stored at -70 ºC.
Gel filtration chromatogram of Cassiopea andromeda crude venom on a Sephadex G-200 (60 × 2.5 cm). A total of 200 mg of dissolved crude venom in 3 ml 0.15 M NaCl (pH 7.2) was applied and eluted with the same buffer. Flow Rate was 0.5 ml/min.
GC–MS of the crude venom and fractions
The analysis of the ATXA dissolved in methanol: hexane (3:2) detected two compounds (ATX A1, A2) using the GC–MS (see supporting information). Their patterns were consistent with ATXA1 (1): 1,2-dicarboxy-3-(4-chlorophenyl)-2,3(1H)-dihydropyrido(1,2-a)benzimidazole; (C19H15ClN2O4); MW: 370., ATXA2 (2): 1,5-diphenyl-1,2-dihydro-3H-1,2,4-triazole-3-thione #; (C14H11N3S); MW: 253. The pattern of ATXB (3) was consistent with 1′H-androst-16-eno[17,16-g]indol-3-ol, acetate (ester), (3á,5à)-; (C27H35NO2); MW: 405.
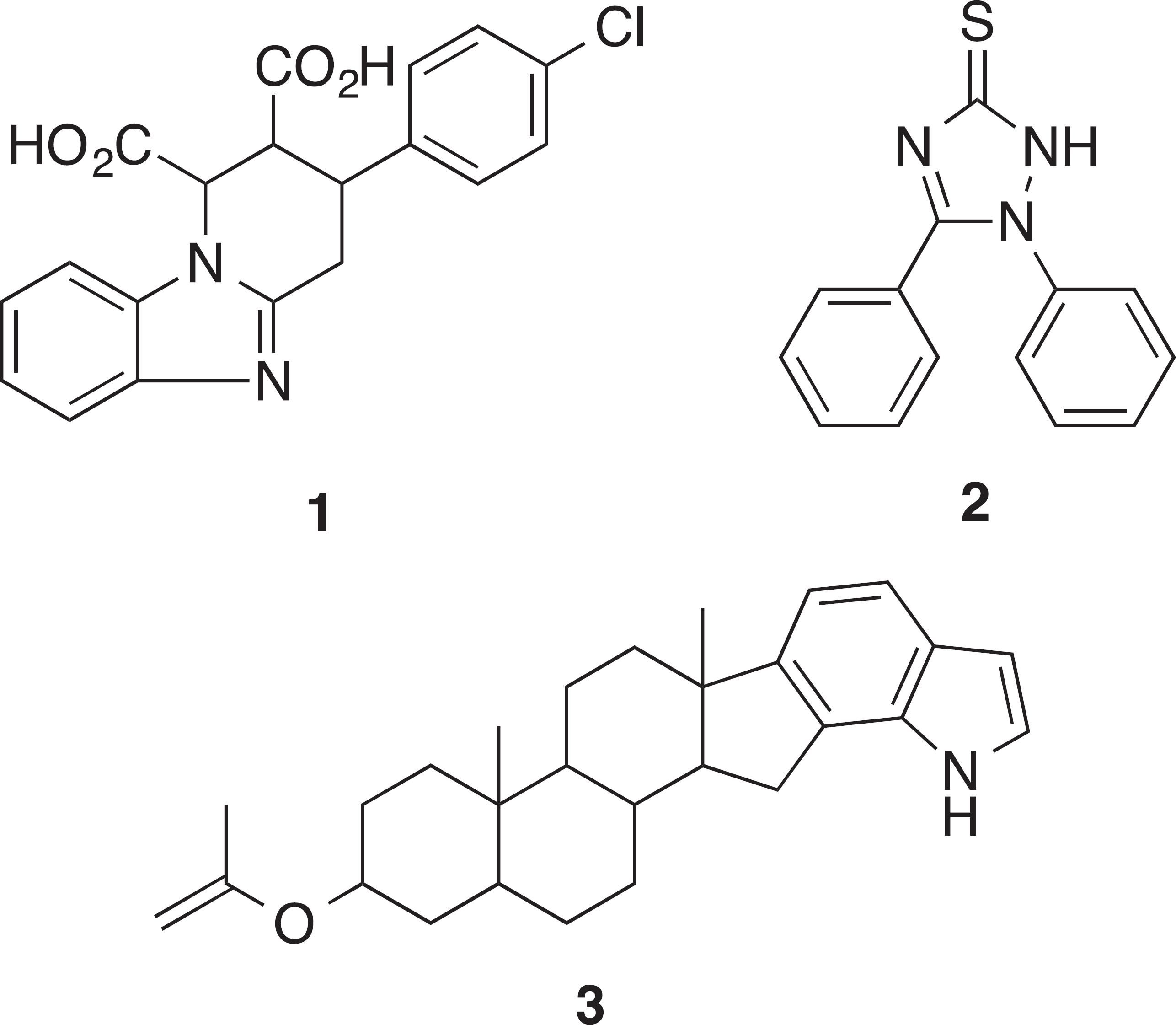
Molecular docking
Table 1 shows the estimated binding energy of the three identified compounds A1, A2, and B. ATXB was the most active compound against rhAChE in comparison with androtoxins A1 and A2 and even the control molecule of galantamine.
The results of docking study for galantamine and the crude venom and isolated compounds from Cassiopea andromeda venom fractions.
The involved residues in the close interaction are listed in Table 2.
The Residues of the isolated compounds from Cassiopea andromeda venom fractions and galantamine in docking study with AChE enzyme.
The investigated compounds were in close contact with two of the three amino acids (Ser203 and His447) of the catalytic triad of AChE through the van der Waals and electrostatic interactions.
ATXB was accommodated within the peripheral site composed by Asp74, Tyr72, Tyr124, Trp286, Phe295, Phe297, Tyr337 and Tyr341 (Fig. 5).
Dockig of the isolated compounds (ATXA1, A2 and B) from Cassiopea andromeda venom fractions and galantamine (as the standard molecule), with AChE enzyme.
The amino nitrogen of this compound formed a hydrogen bond with the hydroxyl group of Tyr341. There were several van der Waals interactions among cycloalkane rings of ATXB, Tyr124 and Tyr337, in addition to the pi-interaction with aromatic ring of Trp286. Close contacts of ATXB with quaternary ammonium binding site residues (such as Trp86) and esteratic site (Gly120 and 121) were found.
NMR spectroscopy
The 1H and 13C NMR spectra of the ATXB dissolved in DMSO were recorded on a Bruker Avance 400. H NMR (400 MHz, DMSO): δ 0.96 (3H, s, CH3), 1.12 (3H, s, CH3), 1.43–1.89 (16H, m, aliphatic), 2.13 (3H, s, OCH3), 2.75 (3H, m, aliphatic), 3.01 (2H, m, COCH2), 5.28 (1H, d, allylic), 6.46 (1H, d, allylic), 6.63 (1H, d, aromatic), 7.34 (1H, d, aromatic). C NMR (100 MHz, DMSO): δ 20.0, 20.9, 21.7, 24.2, 27.7, 28.5, 29.0, 33.2, 33.3, 35.7, 36.0, 36.6, 37.7, 40.9, 43.0, 48.9, 50.3, 74.7, 102.7, 122.6, 125.4, 127.1, 127.8, 130.6, 137.0, 150.2, 170.3 (see supporting information).
In this study, we found that the venom of C. andromeda contained ATXB, the structure of which was elucidated as 1′H-androst-16-eno[17,16-g]indol-3-ol, acetate (ester), (3á,5à)-. This steroidal alkaloid showed a strong AChE inhibitory activity. This compound is the second isolated marine-derived alkaloid steroid with inhibition against AChE.
More than 35 alkaloids have been examined as inhibiting ligands of AChE (Moraga et al., 2016). The cholinesterase-inhibiting activities of steroidal alkaloids from Fritillaria imperialis (Atta-Ur-Rahman et al., 2002), Sarcococca saligna (Zaheer-Ul-Haq et al., 2003), Sarcococca hokeriana (Choudhary et al., 2005), and Holarrhena pubescens (Cheenpracha et al., 2016) have been reported.
Although marine organisms including jellyfishes have been the subject of investigations to determine novel compounds with acetylcholine-inhibiting activity, one report was conducted in the scientific literature on marine-derived steroid alkaloids with AChE-inhibiting activity (Langjae et al., 2007). Langjae et al. (2007) isolated a new stigmastane-type steroidal alkaloid, a 4-acetoxy plakinamine, from the sponge Corticium sp. The comparison of this marine-derived non-pregnane-type steroid with a closely related steroidal alkaloid isolated from Sarcococca showed high potency in its AChE-inhibiting activity. On the contrary, this marine-derived steroid expressed an unusual mixed-competitive mode of enzyme inhibition in the inhibiting mode of most Sarcococca alkaloids.
Zaheer-Ul-Haq et al. (2003) investigated the AChE interactions with fifteen steroidal alkaloids isolated from S. saligna. They found that the ligands bind to the aromatic gorge of their steroid backbone area of the enzyme through the hydrophobic six-membraned ring A. These interactions reduce flexibility at the gorge without externally altering the enzyme structure. According to their docking studies, the AChE inhibitory activities of the ligands mainly exhibited a noncompetitive character (Choudhary et al., 2005).
In the present study, we selected the rhAChE for the molecular docking study because of its more accurate results than Torpedo AChE (Cheung et al., 2012). ATXB was revealed to be active against rhAChE, and its binding energy was greater than that of galantamine.
The most remarkable feature of AChE is a deep and narrow gorge. The catalytic site of AChE is located at this gorge known as the catalytic triad, which is composed of His447, Glu334, and Ser203. This part is considered crucial because of its enzymatic activity, in which acetylcholine is hydrolyzed to acetic acid and choline (Sussman et al., 1991). ATXB produced a close contact with two of the three amino acid residues of the catalytic triad (Ser203 and His447) through van der Waals and electrostatic interactions in the docking study.
A second or peripheral site composed of numerous aromatic site chains that extend beyond Tyr337 at the catalytic/peripheral site interface to the entrance of gorge contributes to the AChE catalytic efficiency (Tara et al., 1998; Szegletes et al., 1999). This site includes Asp74, Tyr72, Tyr124, Trp286, Phe295, Phe297, Tyr337, and Tyr341; Asp74 is responsible for acetylcholine recognition. ATXB was accommodated within this hydrophobic pocket, and the amino nitrogen of this compound formed a hydrogen bond with the hydroxyl group of Tyr341. Several van der Waals interactions were observed among the cycloalkane rings of ATXB, Tyr124, and Tyr337 aside from the pi-interaction with the aromatic ring of Trp286. Thus, the large hydrophobic skeleton of ATXB resulted in hydrophobic interactions with the active sites of AChE. The binding of this compound to both the peripheral and catalytic sites involved extensive protein–ligand contacts. The non-classical CH⋯O hydrogen bond interaction between the hydroxyl group of Asp74 and CH of the cycloalkane ring of ATXB could be involved. Furthermore, the occurrence of the close contact of ATXB with the quaternary ammonium binding site residues (e.g., Trp86) and the esteratic site (Gly120 and 121) confirmed that this compound localized in the active domain of the enzyme.
The biological activities of ATXB have not been studied yet. However, androstanol, its closely related steroid, has been known as a neurosteroid (Kaminski et al., 2006). Neurosteroids are defined as a class of endogenous steroids synthesized within the brain and modulate neurons and glial activity by rapid non-genomic actions (Reddy, 2010; Tvrdeić and Poljak, 2016).
The C19 16-unsaturated steroid androstanol (5α-androst-16-en-3α-ol) has behavioral and social responses in humans through its neuroactivities (Reddy, 2010; Tvrdeić and Poljak, 2016). It is regarded as a potent steroidal pheromone. However, its mechanism of action remains unknown (Gower and Ruparelia, 1993; Grammer et al., 2005). In examining the sub-acute toxicity of the crude venom of C. andromeda, we found that the sexual activities of the experimental rats increased several minutes after the injections (Mohebbi et al., 2016). This interesting finding implies the presence of a pheromone-like activity of the ATXB content of the venom. The bioregulatory functions of steroidal molecules in the phylum Cnidaria, including the Scyphozoa class (“true” jellyfishes), are not elucidated. The identified sex steroids in cnidarian tissues not only have a defensive role but are also likely to serve an endocrine-like signaling role in regulating gametogenesis and/or spawning (Tarrant, 2005). The concentrations of these sex steroids are variable in different seasons in both sexes in the Anthozoa class (e.g., corals and anemones) of the cnidarians. Polar steroids have been speculated to serve as chemical cues or water-borne pheromones to trigger coral spawning (Tarrant, 2005; Tarrant, 2007). These speculations prompt the suggestion that the presence of ATXB in the venom of C. andromeda might play a role as a water-borne pheromone aside from its defensive role.
The prototypic neurosteroids are positive allosteric modulators of GABA-A receptors with diverse pharmacological effects, such as anti-seizure and anti-anxiety activities (Reddy, 2010). Kaminski et al. (2006) showed that androstenol acts as a GABA-A receptor modulator and that its activity is comparable with those of other protypic neurosteroids. ATXB is reduced at the 5- and 3-positions of the A-ring, and the substituents at these positions are in the α-configuration. The potent neurosteroids that are positive modulators of the GABA-A receptor have similar stereochemistry at these critical positions. The 3β-epimer of androstenol does not have modulatory effects on the GABA-A receptors (Kaminski et al., 2006). In the structural homology between ATXB and androstenol, the neurosteroid-like activity of ATXB as a GABA-A receptor modulator should be investigated. Therefore, further studies are required to determine whether ATXB is a potential modulator of GABA-A. The positive answer to this question will open new frontiers in the treatment of epilepsy.
Conclusions
The isolated neurosteroidal alkaloid androtoxin B, from C. andromeda was a potent antiAChE agent with strong binding to both the catalytic and peripheral sites of AChE that correlated well with the experimental data. Further studies are required to determine whether ATXB could be a potential treatment for Alzheimer's disease.
Ethical disclosures
-
Protection of human and animal subjects. The authors declare that no experiments were performed on humans or animals for this study.
-
Confidentiality of data. The authors declare that no patient data appear in this article.
-
Right to privacy and informed consent. The authors declare that no patient data appear in this article.
Acknowledgments
Authors gratefully acknowledge the help and financial support provided by Bushehr University of Medical Sciences and Health Services, Bushehr- Iran. The project was partly supported by Iran National Science Foundation (Research Chair Award N. 95/INSF/44913).
Appendix A Supplementary data
Supplementary data associated with this article can be found, in the online version, at doi:10.1016/j.bjp.2018.06.002.
References
- Ahmed, F., Ghalib, R.M., Sasikala, P., Ahmed, K.K.M., 2013. Cholinesterase inhibitors from botanicals. Pharmacogn. Rev. 7, 121-130.
- Akhtar, M.N., Choudhary, M.I., Tsuda, Y., Sener, B., Khalid, A., Parvez, M., 2002. New steroidal alkaloids from Fritillaria imperialis and their cholinesterase inhibiting activities. Chem. Pharm. Bull. 50, 1013-1016.
- Beedessee, G., Ramanjooloo, A., Surnam-Boodhun, R., van Soest, R.W., Marie, D.E., 2013. Acetylcholinesterase-inhibitory activities of the extracts from sponges collected in mauritius waters. Chem. Biodivers. 10, 442-451.
-
Birks, J., 2006. Cholinesterase inhibitors for Alzheimer's disease. Cochrane Database Syst. Rev. 1, http://dx.doi.org/10.1002/14651858, CD005593.
» http://dx.doi.org/10.1002/14651858 - Bloom, D.A., Burnett, J.W., Alderslade, P., 1998. Partial purification of box jellyfish (Chironex fleckeri) nematocyst venom isolated at the beachside. Toxicon 36, 1075-1085.
- Bradford, M.M., 1976. A rapid and sensitive method for the quantitation of microgram quantities of protein utilizing the principle of protein-dye binding. Anal. Biochem. 72, 248-254.
- Brinkman, D., Burnell, J., 2007. Identification, cloning and sequencing of two major venom proteins from the box jellyfish, Chironex fleckeri Toxicon 50, 850-860.
- Burnett, J.W., Long, K.O., Rubinstein, H.M., 1992. Beachside preparation of jellyfish nematocyst tentacles. Toxicon 30, 794-796.
- Cheenpracha, S., Jitonnom, J., Komek, M., Ritthiwigrom, T., Laphookhieo, S., 2016. Acetylcholinesterase inhibitory activity and molecular docking study of steroidal alkaloids from Holarrhena pubescens barks. Steroids 108, 92-98.
- Cheung, J., Rudolph, M.J., Burshteyn, F., Cassidy, M.S., Gary, E.N., Love, J., Franklin, M.C., Height, J.J., 2012. Structures of human acetylcholinesterase in complex with pharmacologically important ligands. J. Med. Chem. 55, 10282-10286.
- Choi, B.W., Ryu, G., Park, S.H., Kim, E.S., Shin, J., Roh, S.S., Shin, H.C., Lee, B.H., 2007. Anticholinesterase activity of plastoquinones from Sargassum sagamianum: lead compounds for Alzheimer's disease therapy. Phytother. Res. 21, 423-426.
- Choudhary, M.I., Devkota, K.P., Nawaz, S.A., Ranjit, R., Atta-ur-Rahman, v., 2005. Cholinesterase inhibitory pregnane-type steroidal alkaloids from Sarcococca hookeriana Steroids 70, 295-303.
- DeLano, W.L., 2002. The PyMOL Molecular Graphics System. DeLano Scientific LLC, San Carlos, CA.
-
Ellithey, M.S., Lall, N., Hussein, A.A., Meyer, D., 2014. Cytotoxic and HIV-1 enzyme inhibitory activities of Red Sea marine organisms. BMC Complement. Altern. Med. 14, http://dx.doi.org/10.1186/1472-6882-14-77
» http://dx.doi.org/10.1186/1472-6882-14-77 - Farrokhnia, M., Nabipour, I., 2014. Marine natural products as acetylcholinesterase inhibitor: comparative quantum mechanics and molecular docking study. Curr. Comput. Aided. Drug. Des. 10, 83-95.
- Ferreira-Vieira, T.H., Guimaraes, I.M., Silva, F.R., Ribeiro, F.M., 2016. Alzheimer's disease: targeting the cholinergic system. Curr. Neuropharmacol. 14, 101-115.
- Gower, D.B., Ruparelia, B.A., 1993. Olfaction in humans with special reference to odorous 16-androstenes: their occurrence, perception and possible social, psychological and sexual impact. J. Endocrinol. 137, 167-187.
- Grammer, K., Fink, B., Neave, N., 2005. Human pheromones and sexual attraction. Eur. J. Obstet. Gynecol. Reprod. Biol. 118, 135-142.
- Holland, B.S., Dawson, M.N., Crow, G.L., Hofmann, D.K., 2004. Global phylogeography of Cassiopea (Scyphozoa: Rhizostomeae): molecular evidence for cryptic species and multiple invasions of the Hawaiian Islands. Mar. Biol. 145, 1119-1128.
- Kaminski, R.M., Marini, H., Ortinski, P.I., Vicini, S., Rogawski, M.A., 2006. The pheromone androstenol (5α-androst-16-en-3α-ol) is a neurosteroid positive modulator of GABAA receptors. J. Pharmacol. Exp. Ther. 317, 694-703.
- Laemmli, U.K., 1970. Cleavage of structural proteins during the assembly of the head of bacteriophage T4. Nature 227, 680-685.
- Langjae, R., Bussarawit, S., Yuenyongsawad, S., Ingkaninan, K., Plubrukarn, A., 2007. Acetylcholinesterase-inhibiting steroidal alkaloid from the sponge Corticium sp.. Steroids 72, 682-685.
- Mirshamsi, M.R., Omranipour, R., Vazirizadeh, A., Fakhri, A., Zangeneh, F., Mohebbi, G.H., Seyedian, R., Pourahmad, J., 2017. Persian Gulf jellyfish (Cassiopea andromeda) venom fractions induce selective injury and Cytochrome C release in mitochondria obtained from breast adenocarcinoma patients. Asian Pac. J. Cancer Prev. 18, 277-286.
- Mohebbi, G.H., Farzadinia, P., Vatanpour, H., Hashemi, A., Vazirizadeh, A., khazaei, Z., Akbarzadeh, S., Mirshamsi, M., Nabipour, I., 2016. A first record on population of the alien venomous jellyfish, Cassiopea andromeda (Forsskål, 1775) (Cnidaria: scyphozoa: Rhizostomea) in the Nayband Lagoon from Bushehr-Iran (Persian Gulf). Ent. Appl. Sci. Lett. 3, 65-71.
- Moraga, F., Hormazábal, E., Venthur, H., Quiroz, A., Mutis, A., 2016. Alkaloids discovery as natural acetylcholinesterase inhibitors, from nature to molecular docking. Rev. Farmacol. 9, 16-25.
- Morris, G.M., Huey, R., Lindstrom, W., Sanner, M.F., Belew, R.K., Goodsell, D.S., Olson, A.J., 2009. AutoDock4 and AutoDockTools4: automated docking with selective receptor flexibility. J. Comput. Chem. 30, 2785-2791.
- Mustafa, M.R., White, E., Hongo, K., Othman, I., Orchard, C.H., 1995. The mechanism underlying the cardiotoxic effect of the toxin from the jellyfish Chironex fleckeri Toxicol. Appl. Pharmacol. 133, 196-206.
- Nabipour, I., Mohebbi, G.H., Vatanpour, H., Vazirizadeh, A., 2017. Hematological parameters on the effect of the jellyfish venom Cassiopea andromeda in animal models. Data Brief 11, 517-521.
- Nabipour, I., Moradi, M., Mohebbi, G.H., 2015. A first record on population of the alien venomous jellyfish, Cassiopea andromeda (Forsskål, 1775) (Cnidaria: scyphozoa: Rhizostomea) in the Nayband Lagoon from Bushehr-Iran (Persian Gulf). J. Chem. Pharm. Res. 7, 1710-1713.
- Othman, I., Burnett, J.W., 1990. Techniques applicable for purifying Chironex fleckeri (box-jellyfish) venom. Toxicon 28, 821-835.
- Peng, J., Yuan, J.P., Wu, C.F., Wang, J.H., 2011. Fucoxanthin, a marine carotenoid present in brown seaweeds and diatoms: metabolism and bioactivities relevant to human health. Mar. Drugs 9, 1806-1828.
- Pilger, C., Bartolucci, C., Lamba, D., Tropsha, A., Fels, G., 2001. Accurate prediction of the bound conformation of galanthamine in the active site of Torpedo californica acetylcholinesterase using molecular docking. J. Mol. Graph. Model. 19, 288-296.
- Radwan, F.F., Burnett, J.W., Bloom, D.A., Coliano, T., Eldefrawi, M.E., Erderly, H., Aurelian, L., Torres, M., Heimer-de la Cotera, E.P., 2001. A comparison of the toxinological characteristics of two Cassiopea and Aurelia species. Toxicon 39, 245-257.
- Reddy, D.S., 2010. Neurosteroids: endogenous role in the human brain and therapeutic potentials. Prog. Brain. Res. 186, 113-137.
- Sanner, M.F., 1999. Python: a programming language for software integration and development. J. Mol. Graph. Model. 17, 57-61.
- Sarkar, A., Irwin, M., Singh, A., Riccetti, M., Singh, A., 2016. Alzheimer's disease: the silver tsunami of the 21st century. Neural. Regen. Res. 11, 693-697.
- Shanmuganathan, B., Sheeja Malar, D., Sathya, S., Pandima Devi, K., 2015. Antiaggregation potential of Padina gymnospora against the toxic Alzheimer's beta-amyloid peptide 25-35 and cholinesterase inhibitory property of its bioactive compounds. PLOS ONE 10, e0141708.
- Sussman, J.L., Harel, M., Frolow, F., Oefner, C., Goldman, A., Toker, L., Silman, I., 1991. Atomic structure of acetylcholinesterase from Torpedo californica: a prototypic acetylcholine-binding protein. Science 253, 872-879.
- Szegletes, T., Mallender, W.D., Thomas, P.J., Rosenberry, T.L., 1999. Substrate binding to the peripheral site of acetylcholinesterase initiates enzymatic catalysis, Substrate inhibition arises as a secondary effect. Biochemistry 38, 122-133.
- Tara, S., Elcock, A.H., Kirchhoff, P.D., Briggs, J.M., Radic, Z., Taylor, P., McCammon, J.A., 1998. Rapid binding of a cationic active site inhibitor to wild type and mutant mouse acetylcholinesterase: Brownian dynamics simulation including diffusion in the active site gorge. Biopolymers 46, 465-474.
- Tarrant, A.M., 2005. Endocrine-like signaling in Cnidarians: current understanding and implications for ecophysiology. Integr. Comp. Biol. 45, 201-214.
- Tarrant, A.M., 2007. Hormonal signaling in cnidarians: do we understand the pathways well enough to know whether they are being disrupted?. Ecotoxicology 16, 5-13.
- Tommonaro, G., García-Font, N., Vitale, R.M., Pejin, B., Iodice, C., Cañadas, S., Marco-Contelles, J., Oset-Gasque, M.J., 2016. Avarol derivatives as competitive AChE inhibitors, non-hepatotoxic and neuroprotective agents for Alzheimer's disease. Eur. J. Med. Chem. 122, 326-338.
- Tvrdeić, A., Poljak, L., 2016. Neurosteroids, GABAA receptors and neurosteroid based drugs: are we witnessing the dawn of the new psychiatric drugs?. E.O.M. 2, 49-60.
-
ViewrLite5.0, Version Jul 11, 2002. Accelrys Inc.: http://www.accelrys.com
» http://www.accelrys.com - Worek, F., Mast, U., Kiderlen, D., Diepold, C., Eyer, P., 1999. Improved determination of acetylcholinesterase activity in human whole blood. Clin. Chem. Acta 288, 73-90.
- Zaheer-Ul-Haq, Z.U., Wellenzohn, B., Liedl, K.R., Rode, B.M., 2003. Molecular docking studies of natural cholinesterase-inhibiting steroidal alkaloids from Sarcococca saligna J. Med. Chem. 46, 5087-5090.
Publication Dates
-
Publication in this collection
Sep-Oct 2018
History
-
Received
4 Feb 2018 -
Accepted
1 June 2018 -
Published
08 July 2018