Abstract
In this talk I first present an overview of Standard Model predictions for CP violation in the neutral B-meson sector. Then, I present a status report from the <img src="http:/img/fbpe/bjp/v30n2/sbgtra.gif" border="0"> experiment at the Stanford Linear Accelerator Center which has been designed especially to measure time-dependent asymmetries whose interpretation in the Standard Model is directly related to the parameters of the Cabibbo-Kobayashi-Maskawa mixing matrix.
The search for time-dependent CP-asymmetries in the neutral B-meson system
Michael D. Sokoloff
Physics Department
University of Cincinnati
Cincinnati, OH, U.S.A.
E-mail: sokoloff@physics.uc.edu
Received 7 January, 2000
In this talk I first present an overview of Standard Model predictions for
CP
violation in the neutral
B
-meson sector. Then, I present a status report from the
![]()
experiment at the Stanford Linear Accelerator Center which has been designed especially to measure time-dependent asymmetries whose interpretation in the Standard Model is directly related to the parameters of the Cabibbo-Kobayashi-Maskawa mixing matrix.
I Introduction
As particle physicists, we study the fundamental constituents of matter and their interactions. Our understanding of these issues is built upon certain principles: that the laws of physics are the same everywhere, that the laws of physics are the same at all times, that the laws of physics are the same in all inertial reference frames, and that the laws of physics should describe how the wave function of a system evolves in time. The last two issues are embodied in the special theory of relativity and in quantum mechanics. While these principles do not tell us what types of fundamental constituents exist, or how they interact, they restrict the types of theories we consider.
Quantum field theories marry the requirements of special relativity and quantum mechanics, giving us a powerful tool to describe fundamental interactions. A general feature of quantum field theories is that for every type of particle in the theory, there must be a corresponding anti-particle with the same mass and lifetime, and opposite charges (electric, weak, strong, etc.).
We say that a theory is invariant under charge conjugation, C , if the laws of physics are precisely the same for a system of anti-particles as for the corresponding system of particles. Similarly, we say that a theory is invariant under parity inversion, P , if the laws of physics remain precisely the same under a coordinate transformation that takes (x, y, z) to (-x, -y, -z) . Note that parity inversion turns left-handed particles (momentum and helicity anti-parallel) into right-handed particles (momentum and helicity parallel). The weak interaction violates both C and P maximally; that is, it allows only left-handed interactions of particles and only right-handed interactions of anti-particles. However, as a very good approximation, the left-handed weak interactions of particles mimic the right-handed weak interactions of anti-particles. The weak interaction is almost invariant under CP.
The electromagnetic and strong nuclear interactions are invariant under CP transformations, and the weak nuclear interaction has been observed to violate CP at a low level ( » 10-3 ), and only in decays of strange mesons which are rare in every day life. However, the universe is observed to be made up overwhelmingly of matter and not of antimatter. Thus, at the most fundamental level we have studied, the interactions of matter and anti-matter are almost the same, yet at the most macroscopic level we see evidence that nature discriminates between matter and anti-matter most powerfully.
II The Standard Model
In the past thirty years, we have developed a Standard Model of particle physics to describe the electromagnetic, weak nuclear, and strong nuclear interactions of constituents in terms of quantum field theories. The prototype for these theories is quantum electrodynamics. Almost 150 years ago, Maxwell replaced the concept of action at a distance with that of fields, and he unified the electric and magnetic forces into a single framework. The electric and magnetic fields are derived from the scalar and vector potentials, which naturally form a Lorentz four-vector, Am . Classical electrodynamics therefore conforms to the requirement that the laws of physics be the same in all inertial frames. Quantum electrodynamics (QED) quantizes Am , and the corresponding particles, which transfer energy and momentum between electrically charged particles, are the photons.
The fundamental strongly interacting particles are called quarks; they come in six flavors: up (u), down (d), charm (c), strange (s), top (t), and bottom (b). Three (u, c, and t) have electric charge + 2/3 e and are referred to as up-type; the other three (d, s, and b) have electric charge -1/3 e and are referred to as down-type. The theory of strong interactions, quantum chromodynamics (QCD), has a more complicated charge structure than does QED. Where QED has only one type of charge, which comes in plus or minus, QCD has three types of charge, whimsically called color, each of which comes with its own anti-charge or anti-color. A quark might be red or blue or green; an anti-quark might be anti-red, anti-blue, or anti-green. The quanta of the theory, which transfer energy and momenta between strongly charged particles, are called gluons. They are analogous to the photons of QED. However, unlike photons, which are electrically neutral, gluons carry strong charge, each having color/anti-color quantum numbers such as red/blue-bar.
Quarks have never been observed as free particles; only color-neutral objects seem to exist as asymptotic states. Color neutrality can be created by combining three quarks with different colors to form particles we call baryons, or by combining a quark and an anti-quark of corresponding anti-color to form particles we call mesons. The most common baryons are the proton, which is a uud combination, and the neutron, which is a udd combination. The most common mesons are the pions which can be neutral or charged. The p+ is a u state. The corresponding strange particle is the K+ which is a u
state. The neutral kaons we observe experimentally are superpositions of K0 and
0 particles which are eigenstates of the weak interaction. The short-lived neutral kaon, the
, is the symmetric superposition of





Weak charged-current interactions differ fundamentally from electromagnetic or strong interactions; the analogues of the photons and gluons associated with this interaction, the W± particles, transfer electric charge as well as energy and momentum. A u-quark can transform into a d-quark by emitting a W+, for example. As a first approximation, the weak charged current interaction couples fermions of the same generation: u couples to d; c couples to s; and t couples to b so that the flavor eigenstates (of the strong interaction) correspond to the weak eigenstates. But the weak interaction does allow transformations across generations.
In the Standard Model, the Cabibbo-Kobayashi-Maskawa (CKM) matrix, V, transforms the flavor eigenstates to weak eigenstates at the quark level
After absorbing 5 relative phases in the quark fields, the CKM matrix can be described in terms of three rotation angles and one complex phase d. It is this (non-zero) phase which leads to CP violation in the Standard Model.
A parameterization introduced by Lincoln Wolfenstein [1] expresses the elements of the CKM matrix in powers of the Cabibbo angle, l, and three other real parameters, A, r, and h:
The phase angle d of the PDG parameterization [2] is the arctangent of h/ r, and the discussion of the CP violation is often expressed in terms of measurements in the r - h plane.
In the Standard Model, the CKM matrix is unitary:
This leads to a series of "unitarity relationships" which can be described in terms of "unitarity triangles" in the r - h plane. The most commonly considered relationship is
This is illustrated in Fig. 1 which is reproduced from the Baar Technical Design Report (TDR) [3] The interior angles of this triangle, a, b, and g, determine CP violating rates which can be measured experimentally. The interior angles of all the possible unitarity triangles are independent of phase convention in the CKM matrix. As is true in the Wolfenstein parameterization, the matrix elements Vcd and Vcb are usually chosen to be purely real so that the apex of the triangle sits at the point (r, h) . The CKM phase allows for the possibility of CP violation in the weak decays of hadrons when two or more amplitudes contribute to the same final state.
An especially interesting series of measurements can be made in the case of B0- 0 mixing with subsequent decays to CP eigenstates. Second order weak charged-current processes, often referred to as box diagram amplitudes, provide a mechanism by which B0 particles oscillate into
0 anti-particles, and vice versa. The Feynman diagram for one set of these amplitudes is illustrated in Fig. 2. Because the weak charged current couples generations, the full amplitude must include contributions from all of the up-type quarks coupling to the b quarks in the Feynman diagram. In the limit that these up-type quarks all have the same mass, the unitarity of the CKM matrix leads to exact cancellation of the box diagram amplitudes. Because the quark masses differ, the cancellation is not complete. The top quark mass is so much greater than the charm and up quark masses that the characteristic mixing time in the neutral B -meson system is about the same as the characteristic decay time. Writing the equation for mixing as
the experimental result is
Because the characteristic mixing and decay times are so similar, observing the mixing is relatively easy experimentally. Were the mixing time much greater than the decay time, the number of mixed events to observe would be much smaller. And were the mixing time much less than the decay time, current detectors would lack the spatial resolution to resolve the oscillations. (This is the case today for BS - S mixing.)
The amplitude for B0 oscillating into 0 has a phase equal and opposite to the phase for
0 oscillating into B0. This leads to the expectation for CP violation in B0-
0 mixing. Consider a B -decay final state f, such as J/Y
, which is available for either B0 or
0. If a B -meson starts life as a B0 it may decay into f either directly, or having oscillated into a
0 first. Similarly, if it starts life as a
0 it may decay into f directly, or having oscillated into a B0 first. The relative phases of the two amplitudes which take B0 to f, one directly and the other via mixing, will change sign when we consider
0 to f. This leads to asymmetries in the decay rates to f. And because the ratio of mixed to unmixed events varies with time, the asymmetry will also vary with time, providing important experimental constraints when interpreting results.
The two amplitudes which interfere are the mixed and unmixed amplitudes (ai and i) to a CP eigenstate. This CP violation does not depend on the strong phase. Interpretation is especially easy if there is only one ai associated with B0 decay to a particular final state. The asymmetries associated with such decays provide direct measurements of the angles a, b, and g.
The algebra is tedious but fairly straight-forward. The time evolution of a B0 or 0 is determined by Schrödinger's Equation:
Diagonalizing the Hamiltonian, Schrödinger's Equation has solutions of the form e-iwt = e-i ( m-ig/2) t with eigenvalues
for the "light" and "heavy" eigenstates
with
The mass and width differences of the eigenstates are usually discussed in terms of the variables
The value of the mass difference variable for the Bd system, xd, is determined to be 0.73 ± 0.04 by the PDG [2]. No experimental evidence exists for a width difference, and theorists generally expect y ~ (10-2) . Thus, the phase of q/p above depends only on M12. In the Standard Model, M12 originates in a box diagram that generates the phase
where we assume a parameterization of the CKM matrix in which Vtb is real.
The flavor eigenstates |B0(t)ñ and |0(t)ñ can be written in terms of the weak eigenstates:
where m º (mH + mL)/2 and G = GH = GL .
For any final state f, there are four decay amplitudes for the pure B0 and 0 states:
The decay rate to a particular final state depends on all the amplitudes which can produce it:
The time-dependent asymmetry becomes
where k º (q/p) (f /
f). For B0 and
0 decays to CP eigenstates in which only one tree-level amplitude contributes,
so that the decay asymmetry becomes
where the superscript is the CP eigenvalue of f.
Two of the most important CP eigenstates will study are B0® Y
(BR » 5 ×10-4), for which [4] (using the Wolfenstein parameterization)
and B0® p+p- (BR » 2 ×10-5), for which [4]
assuming only tree-level amplitudes are significant. For B0® Y, the penguin amplitudes have the same weak phase as does the tree-level amplitude, so measuring the asymmetry still allows the direct extraction of sin2 b. For B0® p+p- the penguin and tree-level weak phases differ, so extracting sin2 a from the asymmetry measurement will require more experimental and theoretical work [4].
Experimentally, DM ×G » 0.7, which makes it possible to observe the time dependencies of these asymmetries. is doing this using asymmetric e+e-® U(4S) ® B0
0 events, as illustrated in Fig. 3. The high energy (e-) ring operates at 9.0 GeV. The low energy (e+) ring operates at 3.1 GeV. Because the mass of the U(4S) is just above threshold for the production of B
pairs, the B -mesons as well as the U(4S) are co-moving along the beam axis with bg = 0.56. The typical separation between the two B decay vertices in an event is 250 mm.
This cartoon illustrates the relationship between the e+e- interaction point and the decay points of the two B-mesons used in measuring time-dependent CP violation. Because the B-mesons are produced approximately at rest in the CM, the vertex separation is primarily along the direction of motion of the CM.
Because the U(4S) is a pure (CP = -1) state, the B0
0 state is coherent until one of the B's decays. At this point, one is a B0 and the other a



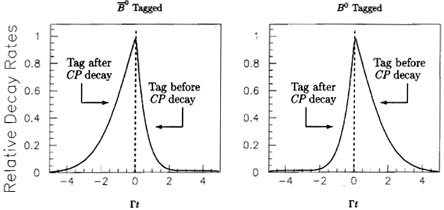


In the Standard Model, the unitarity angles a, b and g are determined by the parameters r and h. These are constrained by data from K° decay (), B and charm decays, and from
-
mixing. In addition, theoretical calculations of hadronic matrix elements are used. The shaded area in Fig. 5, taken from the review article by Richman and Burchat [5], corresponds to that allowed for the apex of the unitarity triangle circa 1995.
Constraints in the Standard Model in the rh plane. The shaded area corresponds to that allowed for the apex of the rescaled unitarity triangle circa 1995 [5].
These measurements indirectly constrain the phase the of CKM matrix. But they do not establish the existence of the phase or establish the unitarity of the CKM matrix. Rather, they assume these features. Measuring the time-dependent CP asymmetries in B0 and BS decay can directly establish the CKM phase as the origin of CP violation. Checking experimentally that a + b + g = 180 ° will test the unitarity of the CKM matrix. Comparing the angles derived by these CP asymmetry measurements with those determined from ratios of branching ratios, the B0 - 0 mixing rate, etc. will provide further tests of the Standard Model.
III The
The experiment has been built at the Stanford Linear Acceleration Center (SLAC) to exploit PEP-II, an asymmetric e+e- collider which operates at the U(4s) resonance. At the peak of this resonance, approximately 25% of all hadronic events are B
events, and of these, approximately half are B0

The silicon vertex tracker has five layers of double-sided silicon microstrip detectors. One side measures the longitudinal coordinate, the other measures the azimuthal coordinate. In cosmic ray data taken before collisions started in the detector, the azimuthal and longitudinal resolutions were measured with r.m.s. values of 15 mm and 19 mm, as observed in Fig. 7. The resolution varies as a function of the angle at which a track traverses the detector. Fig. 7 shows how the resolution in the first layer of the SVT varies as a function of laboratory angle for a single run, and compares the measurements with Monte Carlo simulations.
This figure shows the azimuthal and longitudinal resolutions in the first layer of the SVT as a function of track angle measured using cosmic ray data before beam was available. Monte Carlo data is compared to real data, showing that the detector performance is approaching design specications.
The drift chamber has 40 layers divided into 10 alternating axial and stereo superlayers. We use a low density 80% He, 20% isobutane gas mixture and aluminum wires. The detector is designed to provide 7% ionization (dE/dx) resolution and 140 mm spatial resolution. Fig. 8 shows the measured spatial resolution as a function of position in a drift chamber cell. The mean value, weighted for how many tracks traverse each part of a cell, is 125 mm, which is better than the design specification. The dE/dx resolution measured in Bhabha events is shown in Fig. 9. The resolution is about 7.5%, which is approaching the design resolution. This will allow good p-K separation up to 700 MeV/c, and good proton identification up to 1.2 GeV/c, as seen in Fig. 10.
Drift chamber resolution is shown as a function of track position within a drift chamber cell. Most tracks occupy regions where the resolution is good, so the average resolution is now 125 mm where the design value was 140 mm.
Specific ionization in the drift chamber from Bhabha scattering events. The design resolution is 7%, so once again we see that the detector performance is rapidly approaching design specifications.
Specific ionization measured in the drift chamber in early summer, 1999. Good p-K separation is observed below 600 MeV/c.
The DIRC is a detector of internally reflected Cerenkov light. Its principle of operation is illustrated in Fig. 11. When a fast charged track traverses a quartz bar (index of refraction » 1.47), it emits Cerenkov light in a cone coaxial with the track's motion. Most of the photons will be totally internally reflected at the interface of the quartz bar and air, and will be transported to a water standoff box where they will be refracted rather than reflected. The image of the cone expands as the photons pass through 1.2 m of water before impinging on an array of photomultiplier tubes. The angle of an individual photon with respect to the charged track is determined by tracing its trajectory backwards, assuming it emerged from the center of the quartz bar and hit the center of the phototube. The cone of light emitted by a single track will transform into a conic section in the array of phototubes, and the Cerenkov angle associated with each track is determined by fitting the Cerenkov angles of the single photons which might be part of its image.
The DIRC measures the Cerenkov angle of light produced by charged particles traversing quartz bars to identify the species of the charged particles. Photons are internally reflected to the end of the quartz bars where they emerge into a water stand-off box and spread out forming conical images on the plane of phototubes.
The Cerenkov angles for backward positrons from Bhabha events is shown in Fig. 12. The observed resolution is 3.0 mrad. The design resolution for such tracks is 2.6 mrad. This detector is rapidly approaching its design specification.
This figure shows the Cerenkov angle measured for tracks from Bhabha scattering events. The observed resolution is about 3.0% where the design specification is 2.6%.
The electromagnetic calorimeter is a critical detector for time-dependent CP-asymmetry measurements. The standard gold-plated channel is B to J/Y, where Y® l+l-. Furthermore, tagging the non-CP B is done most frequently and most cleanly with high pT leptons. In addition, it allows us to identify p0® gg and h ® gg candidates for use in reconstructing B and D decays. A clear p0 peak is observed in the gg invariant mass spectrum shown in Fig. 13.
A clear p0 peak emerges when we require each photon have energy greater than 50 MeV and the di-photon pair have energy greater than 500 MeV.
To search for B ® J/Y, we first look at inclusive distributions to see the constituents. Fig. 14 shows a clean inclusive
peak in a p+p- invariant mass distribution. The two tracks were required to form a good vertex, and the summed momentum vector was required to point back to the beam spot. Fig. 15 shows inclusive e+e- and m+m- distributions in which we see J/Y signals. The data are very much preliminary, but the efficiencies are roughly what we expect at this stage of the experiment.
To select candidate B ® J/Y combinations, we calculate the invariant mass of four-track candidates where the constituents form J/ Y and
candidates consistent with coming from a common vertex. We calculate the invariant mass of the J / Y
candidate, and also the difference between this combination's energy in the e+e- center-of-mass and the energy expected for a B -meson produced there, DE . The masses and energy differences for the candidates from very early data are plotted in Fig. 16. The box in the scatter plot spans ±3 standard deviations in DE and in invariant mass. It occupies about 10% of the area of the scatter plot. Two entries are observed outside the box, leading to an estimate of roughly 0.2 background events inside the box. Two events are observed. Based on crude luminosity measurements and our current understanding of reconstruction efficiencies, we expect somewhere between 1 and 2 signal events. 4The data is therefore consistent with our expectations and with our having observed at least one B ® J/Y
decay. We see similar evidence for B0® D*-p+ (another 2 events inside the signal region where background is estimated to be a small fraction of an event). This gives us confidence that the detector is working and our goals for the first year of running are realistic.
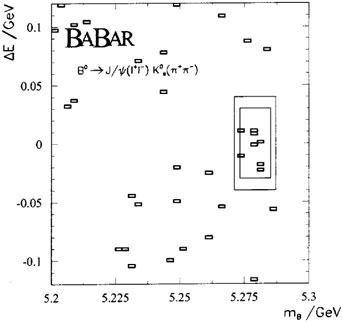

The design luminosity of PEP-II is 3 ×1033 cm-2 sec-1, which should produce 30 ×106
b pairs per year. At the time of this conference,

Over the next three to five years, should be able to measure CP violation using high statistics samples in many decay modes (thousands in B ® J/Y
and thousands in other decay modes). This should allow us to test the Standard Model: if the value of sin2 b we determine does not accord with the range allowed in the Standard Model by other types of measurements, as illustrated in Fig. 5, we will have evidence of new physics; if the value of sin2 b measured in one decay mode does not accord with that measured in another decay mode, we will have evidence of new physics; If the values of a, b, and g that we determine are not consistent with a + b + g = 180°, then we will have evidence of new physics. And if all of our measurements are self-consistent within the framework of the Standard Model, we will have made precision measurements of the relative phases of the CKM matrix elements. Whatever the outcome, our data will help guide the quantitative understanding of CP violation.
It is my pleasure to thank the organizers of this conference for inviting me to participate and to present a status report on the experiment. As is true so often today in experimental high energy physics, the success of the project is the result of very many people working very hard, and my part in the experiment has been very small. In addition to the hundreds of participants in
itself, we are indebted to all those who made the PEP-II accelerator work so well, so quickly, and who continue to push its limits. They are heroes. This work was supported in part by the U.S. National Science Foundation.
[3] The Collaboration, B. Boutigny et al.,
Technical Design Report, SLAC-R-95-457 (March, 1995).
- [1] L. Wolfenstein, Parameterization of the Kobayashi-Maskawa matrix, Phys. Rev. Lett. 51, 1945 (1983).
- [2] Particle Data Group, C. Caso et al., Review of Particle Properties, The European Physical Journal C 3, 1 (1998).
- [4] Y. Nir and H. Quinn, CP violation in B physics, Ann. Rev. Nucl. Part. Sci. 42, 211 (1992), and references therein.
- [5] Jeffrey D. Richman and Patricia R. Burchat, Leptonic and semileptonic decays of charm and bottom hadrons, Rev. Mod. Phys. 67, 893 (1995).
Publication Dates
-
Publication in this collection
07 Jan 2002 -
Date of issue
June 2000
History
-
Received
07 Jan 2000